ANNLab Research
Atomically Precise Graphene Nanoribbon Transistors for High-Performance Logic Technologies
Graphene nanoribbons (GNRs), synthesized through on-surface methods via bottom-up approaches, are a chemically tunable class of low-dimensional quantum materials with promising semiconducting properties. Theoretically, GNRs offer high charge mobility and current-carrying capabilities, making them strong candidates for channel materials in field-effect transistors (FETs). GNR-based FETs could exceed the performance and energy efficiency of conventional silicon transistors while enabling novel functionalities beyond current semiconductor technologies. However, a significant gap remains between their theoretical promise and experimental device performance. To address this, we are focused on deepening our understanding of charge transport mechanisms in atomically precise GNRs, with particular emphasis on contact and dielectric interface engineering, bandgap and edge-state tuning, and refinement of device and chip-level architectures to improve carrier injection and transport. In parallel, we are developing scalable integration strategies, including wafer-scale synthesis with precise alignment and pitch control, as well as etch-free dry transfer and direct growth on insulating substrates to minimize transfer-related artifacts and preserve interfacial quality. We are also investigating nanoscale electronic behavior using in situ scanning probe techniques and exploring plasmonic properties through electron microscopy–based methods. To complement our experimental efforts, we are testing and developing modern computational tools, including machine learning models and large language models (LLMs), to interpret complex datasets, mine published literature, and accelerate experimental planning and process optimization. Through this integrated approach, we aim to enable the reliable and scalable incorporation of GNRs into high-performance logic transistors and advanced nanoelectronic systems. One of our most ambitious milestones is to demonstrate the world’s first fully functional computer built entirely from a GNR-based microprocessor, capable of operating beyond the limitations of silicon technology, leveraging both classical and quantum principles, and proudly displaying on its screen: “Hello, world. I am the first graphene computer.”
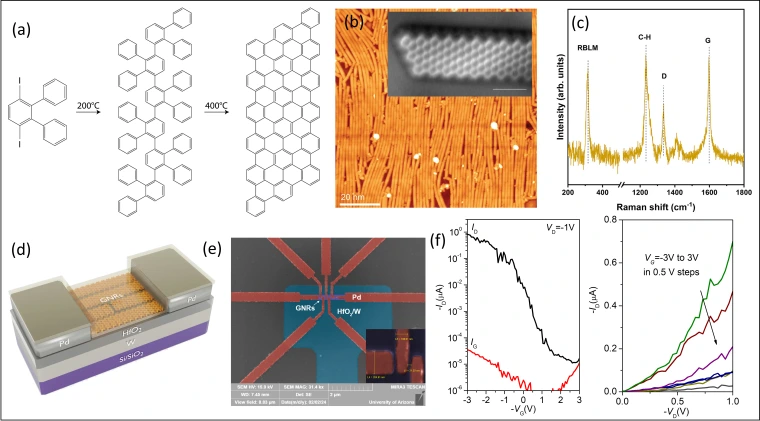
(a) On-surface synthesis of nine-atom-wide armchair graphene nanoribbons (9-AGNRs) on an Au(111)/mica substrate using DITP precursor molecules. (b) STM image of 9-AGNRs on Au(111)/mica (inset: nc-AFM image of a single ribbon; scale bar: 1 nm). (c) Raman spectrum of 9-AGNRs on Au(111)/mica. (d) Schematic of a 9-AGNR field-effect transistor (GNRFET) with a local back gate. (e) SEM image of a fabricated 9-AGNRFET device (inset: high-magnification view of the Pd contacts). (f) Electrical performance of a representative GNRFET: transfer characteristics (ID–VGS, left) and output characteristics (ID–VDS, right). (Adapted from our publications)
Topological Acoustic Wave Devices for Advanced Radio-Frequency and Sensing Technologies
Topological acoustic (TA) wave devices are emerging as a promising platform for next-generation radio-frequency (RF) technologies and advanced sensing systems. Drawing on principles from topological physics, these devices utilize engineered band structures and topological protection to enable robust, low-loss propagation of acoustic waves, even in the presence of structural disorder or fabrication imperfections. Through careful design of surface periodicity and delay line geometries, it is possible to manipulate Bragg reflections and phononic bandgaps for frequency-selective attenuation and dynamic control of wave propagation. This research involves a full-cycle development approach, including digital mask design, photolithography patterning, fabrication on piezoelectric substrates, and experimental characterization using tools such as scanning electron microscopy, optical microscopy, and S-parameter analysis. Surface acoustic wave (SAW) devices are a central focus, with designs incorporating interdigital transducers (IDTs) and variable finger spacings to enable on-chip frequency control. Additional work explores the use of femtosecond laser ablation to engineer periodic surface features, such as trench patterns, that support the formation of acoustic bandgaps and tailored stop-band behavior. Computational modeling, including finite element simulations, is integrated with experimental feedback to refine design parameters such as trench width, pitch, and laser processing conditions. This iterative process supports the development of reconfigurable acoustic architectures capable of high-performance signal control. Beyond RF applications, TA wave platforms are being investigated for their potential in probing quantum phenomena within biological environments. These systems could offer new insights into coherence, entanglement, and quantum-assisted energy transport, with implications for understanding biomolecular function and the quantum nature of life. By combining concepts from topological physics, acoustic engineering, and quantum biophysics, this interdisciplinary research aims to establish multifunctional SAW-based platforms that advance scalable signal processing and communication systems.
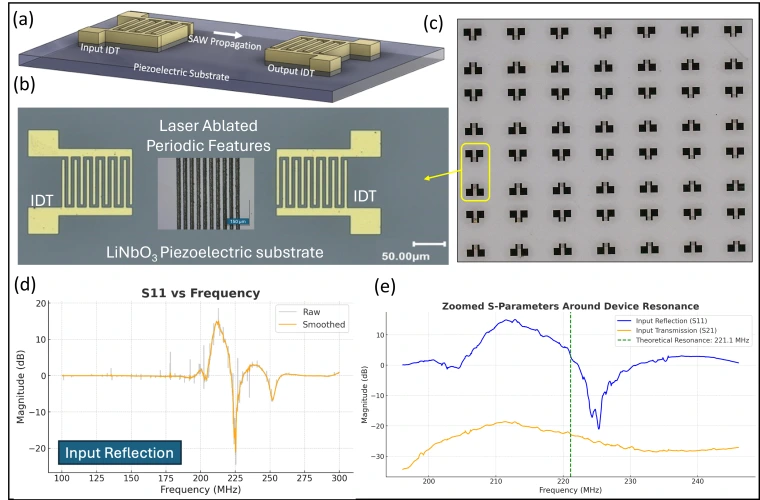
(a) Schematic illustration of a surface acoustic wave (SAW) device consisting of input and output interdigitated transducers (IDTs) on a piezoelectric substrate, enabling guided SAW propagation. (b) Optical micrograph of a fabricated SAW device on a lithium niobate (LiNbO₃) substrate, with laser-ablated periodic features introduced between the IDTs to induce acoustic bandgap effects. (c) Optical image of a centimeter-scale chip with an array of SAW devices, each designed for different operating frequencies. (d) Full S-parameter sweep from 100 to 300 MHz, showing input reflection characteristics; both raw and smoothed data are presented. (e) Zoomed-in view of the S-parameters near the device's theoretical resonance at 221.1 MHz, showing input reflection and forward transmission behavior. (Adapted from Howard Yawit's presentation slides)
Semiconductor Properties of Chalcopyrite and Their Role in Copper Leaching Efficiency
Chalcopyrite (CuFeS₂), a widely occurring copper-bearing mineral, presents an ongoing challenge in the mining industry due to its low leaching behavior. This limitation affects both the efficiency and sustainability of conventional copper extraction processes. It has been hypothesized that the semiconducting properties of chalcopyrite play an important role in influencing its leaching behavior. Understanding its semiconducting behavior offers a promising route to address these limitations. This work focuses on the electrical characterization of chalcopyrite samples from various geological sources, aiming to explore correlations between their electronic transport properties and their response to leaching conditions. Using a Hall measurement setup, key parameters such as carrier concentration, mobility, resistivity, and conduction type are systematically evaluated Standardized procedures for sample preparation and data collection are being developed to ensure consistency and reproducibility. Measurements are conducted under temperature-controlled conditions to study electrical transport behavior across a range of relevant environments. Complementary microscopy and microstructural analysis are used to relate electrical characteristics to features such as grain size, surface morphology, and compositional uniformity. By integrating approaches from solid-state physics, materials science, and mineral processing, this research aims to inform strategies that improve copper extraction processes through enhanced efficiency and performance.
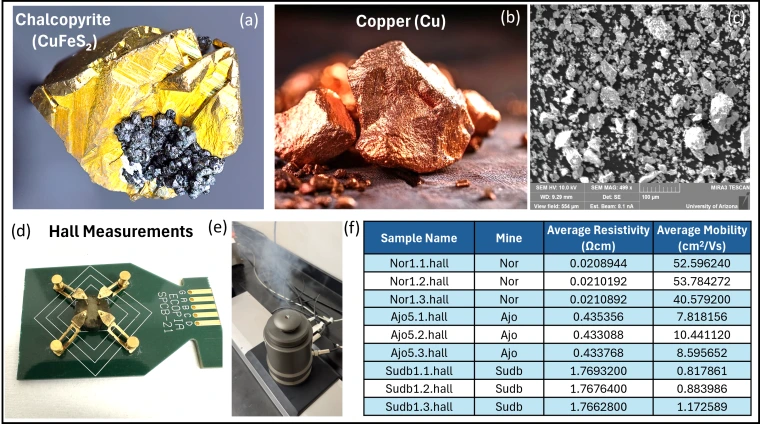
(a) Photograph of chalcopyrite and (b) raw copper metal. (c) Scanning electron microscopy (SEM) image showing the granular microstructure of a chalcopyrite sample (d) Hall bar device used for in-plane transport measurements of electronic properties. (e) Cryogenic setup for temperature-dependent Hall measurements. (f) Table summarizing the average resistivity and mobility values obtained from Hall measurements of chalcopyrite samples sourced from different mine locations. (Adapted from Julian Battaglia's presentation slides)
Emerging Ideas and Out-of-the-Box Science
In addition to our flagship projects outlined above, ANNLab is actively exploring a range of emerging and interdisciplinary research directions, many of which are in early discovery phases or developed through collaborative initiatives. One growing focus area is the investigation of radiation-related properties of low-dimensional materials. We are studying how their unique quantum and structural characteristics can be harnessed for radiation sensing and for developing lightweight, resilient shielding solutions in extreme environments such as space or nuclear facilities. We are also advancing the synthesis of single-crystalline, atomically thin 2D dielectrics, tailored for high breakdown strength, minimal defect density, and precise quantum control. These materials form the foundation for next-generation nanoelectronic and quantum platforms, enabling high-fidelity interfaces and devices with minimal leakage and loss. Beyond traditional applications, our research increasingly explores unconventional and cross-disciplinary ideas. For instance, we are investigating how topological acoustic devices might be used as biological sensors, capable of detecting subtle vibrations or frequencies in natural systems. Such approaches could, one day, help us understand the “language” of animals or decode biological signals in entirely new ways.